Originally
published In Press as doi:10.1074/jbc.M408531200
on November 22, 2004
J. Biol. Chem., Vol. 280, Issue 8, 6950-6959, February 25,
2005
Acceleration of Glutathione Efflux and Inhibition of
-Glutamyltranspeptidase Sensitize Metastatic B16 Melanoma Cells to
Endothelium-induced Cytotoxicity*
María Benlloch
, Angel Ortega
¶,
Paula Ferrer
, Ramón Segarra
,
Elena Obrador
,
Miguel Asensi
,
Julián Carretero||, and José M. Estrela
**
From the
Departamento de Fisiología, Universidad de Valencia, 46010
Valencia, Spain and the ||Centro Nacional de Investigaciones
Oncológicas, 28029 Madrid, Spain
Received for publication, July 28, 2004 , and in revised form, November 19,
2004.
 |
ABSTRACT |
Highly
metastatic B16 melanoma (B16M)-F10 cells, as compared with the low
metastatic B16M-F1 line, have higher GSH content and preferentially
overexpress BCL-2. In addition to its anti-apoptotic properties,
BCL-2 inhibits efflux of GSH from B16M-F10 cells and thereby may
facilitate metastatic cell resistance against endothelium-induced
oxidative/nitrosative stress. Thus, we investigated in B16M-F10 cells
which molecular mechanisms channel GSH release and whether their
modulation may influence metastatic activity. GSH efflux was
abolished in multidrug resistance protein 1 knock-out (MRP-/-1)
B16M-F10 transfected with the Bcl-2 gene or in MRP-/-1
B16M-F10 cells incubated with L-methionine, which
indicates that GSH release from B16M-F10 cells is channeled through
MRP1 and a BCL-2-dependent system (likely related to an L-methionine-sensitive GSH carrier previously detected
in hepatocytes). The BCL-2-dependent system was identified as the
cystic fibrosis transmembrane conductance regulator, since monoclonal
antibodies against this ion channel or H-89 (a protein kinase
A-selective inhibitor)-induced inhibition of cystic fibrosis
transmembrane conductance regulator gene expression completely
blocked the BCL-2-sensitive GSH release. By using a perifusion system
that mimics in vivo conditions, we found that GSH depletion in
metastatic cells can be achieved by using Bcl-2 antisense
oligodeoxynucleotide- and verapamil (an MRP1 activator)-induced
acceleration of GSH efflux, in combination with acivicin-induced
inhibition of
-glutamyltranspeptidase (which limits GSH synthesis by
preventing cysteine generation from extracellular GSH). When applied
under in vivo conditions, this strategy increased tumor
cytotoxicity (up to
90%)
during B16M-F10 cell adhesion to the hepatic sinusoidal
endothelium.
 |
INTRODUCTION |
B16
melanoma (B16M)1
cells with high glutathione (GSH;
-glutamylcysteinylglycine) content show higher metastatic
activity in the liver than those with low GSH content (1). The liver is
a common site for metastasis development, and we demonstrated that
GSH protects B16M cells against nitrosative and oxidative stress in
the murine hepatic microvasculature (2, 3). The concept
that high GSH content status is an important factor for metastasis
progression was strongly supported by the fact that metastatic B16M
cell survival and growth can be enhanced by directly increasing their
GSH content with GSH ester, which readily enters the cell and
delivers free GSH (4, 5). In
consequence, the maintenance of high intracellular levels of GSH
appears critical for metastatic cells to survive intravascularly and
to progress extravascularly.
Multidrug and/or radiation resistance, which are characteristic
features of malignant tumors, frequently associate with high
GSH content in the cancer cells (6). Efflux of GSH
and GSH S-conjugates from different mammalian cells is
mediated by multidrug resistance proteins (MRP), among which MRP1 and
MRP2 have been characterized at the functional level as ATP-dependent
pumps with broad specificity for GSH and glucuronic or sulfate
conjugates (7–10).
Multidrug resistance frequently associates with the overexpression
of P-glycoprotein and/or MRP1 (11), both
functioning as pumps that extrude drugs from tumor cells. GSH
depletion induced by L-buthionine-(SR)-sulfoximine (BSO), a specific
inhibitor of
-glutamylcysteine synthetase (the rate-limiting step in GSH
biosynthesis) (12),
resulted in a complete reversal of resistance to anticancer drugs
of different cell lines overexpressing MRP1 but had no effect on
P-glycoprotein-mediated multidrug resistance (13). Most
interestingly, cancer cells can release GSH through MRP1 even in the
absence of cytotoxic drugs (7).
In a recent study we demonstrated that B16M-F10 cells with a high
metastatic potential overexpress BCL-2, show an increase in
intracellular GSH content, show no change in the GSH synthesis rate,
but show a decrease in GSH efflux (5). This study
also provides evidence that BCL-2 can directly inhibit GSH
export, thereby accounting for the increase in intracellular GSH.
Moreover, it demonstrates that GSH depletion and BCL-2 antisense
therapy can sensitize cells to TNF-
-induced
apoptotic death. In consequence, it is plausible that BCL-2, in
addition of its anti-apoptotic properties (14), may also
increase metastatic cell resistance against oxidative/nitrosative
stress by preserving intracellular GSH. In fact, GSH efflux prior or
during apoptosis appears an essential regulator of the apoptotic
killing mechanism (15).
Because GSH efflux from B16M cells can be increased by using
Bcl-2 antisense oligodeoxynucleotides (Bcl-2-AS) (5), and
possibly by using different MRP1 regulators, the aim of the present
report was to investigate whether the rate of efflux may become
an important factor regulating intracellular GSH content and
thereby metastatic cell survival.
 |
EXPERIMENTAL PROCEDURES |
Culture
of B16M-F10 Cells—Murine B16M-F10 cells (from the ATCC, Manassas,
VA) were cultured in Dulbecco's modified Eagle's medium (DMEM; Invitrogen), pH
7.4, supplemented with 10% fetal calf serum (Invitrogen), 10
mM HEPES, 40 mM
NaHCO3, 100 units/ml penicillin, and 100 µg/ml
streptomycin (4).
Cell integrity was assessed by trypan blue exclusion and leakage
of lactate dehydrogenase activity (4).
GSH and GSSG—GSH was measured by the glutathione
S-transferase reaction and GSSG by high pressure liquid
chromatography (4).
Total glutathione (GSH + 2GSSG) was determined by a kinetic
assay in which a catalytic amount of GSH or GSSG and glutathione
reductase cause the continuous reduction of
5,5'-dithiobis-2-nitrobenzoic acid (Sigma) by NADPH (16). GSH
monoisopropyl(glycyl) ester was prepared as described previously (17).
Bcl-2 Gene Transfer and BCL-2 Analysis—The Tet-Off gene
expression system (Clontech) was used, as in Ref. 5, to insert
the mouse Bcl-2 gene and for transfection into B16M cells
following the manufacturer's instructions. BCL-2 protein was
quantitated in the soluble cytosolic fraction by enzyme immunoassay
(18)
using a monoclonal antibody-based assay from Sigma (1 unit of
BCL-2 was defined as the amount of BCL-2 protein in 1,000
nontransfected B16M cells).
Cellular BCL-2 protein levels were also analyzed, as recently
reported (5), by flow
cytometry using an EPICS PROFILE II (Coulter Electronics, Hialeah,
FL), at 488 nm and 250 milliwatts. Cellular suspensions were diluted
to
250,000 cells/ml. Primary monoclonal anti-BCL-2 from mouse
followed by biotin-conjugated goat anti-mouse IgG and
phycoerythrin-labeled streptavidin (Sigma) were used. BCL-2 protein
levels were expressed as arbitrary fluorescence units (
FL1). Cell viability in these experiments was determined
with propidium iodide (final concentration 10 µM,
Molecular Probes, Eugene, OR).
Electroporation and Selection of B16M-F10 Multidrug Resistance
Protein Knock-out Clones—Murine MRP genomic sequences were
isolated from a
Charon
35 phage library constructed with DNA from mouse strain C57BL/6J. The
preparation of the gene-targeting construct, electroporation into
tumor cells (Bio-Rad), and selection and screening of the clones,
first by PCR analysis and then by DNA blot analysis, were carried out
as described previously (19). The
multidrug resistance associated protein-1 or -2 knock-out clones
(MRP-/-1 and MRP-/-2) were obtained by exposing a single knock-out
clone to high concentrations of G418 for 2 weeks.
Treatment of B16M-F10 Cells with Oligodeoxynucleotides—B16M-F10
cells were incubated, as described previously (5), in the
presence of Bcl-2 antisense phosphorothioate-derivatized
oligodeoxynucleotides (Bcl-2-AS) from Eurobio (Les Ulis,
France). Fresh oligodeoxynucleotides were added every day, starting
24 h after cell plating, as 1:1 complexes with the Lipofectin
transfection reagent (Invitrogen).
The sequence of Bcl-2-AS corresponding to the mouse Bcl-2
translation initiation codon site and extending 3' downstream for a
total of 18 bases was 5'-TCTCCCGGCTTGCGCCAT-3' (20). A 2-base
Bcl-2 mismatch oligodeoxynucleotide (5'-TCTCCCGGCATGTGCCAT-3')
was used as control.
Quantitative Determination of the Plasma Membrane Potential—
Plasma membrane potential (PMP) was measured using a standard
technique (21). B16M-F10
cells were cultured for 24 h, as described above, but seeded at 5
x 104 cells/cm2 in glass
Petri dishes. Culture dishes were mounted on a tube-focusing
microscope (Nikon, Tokyo, Japan). Intracellular measurements were
performed, at 25 °C, with glass micropipettes filled with 3 M KCl and with a 20-megohm DC resistance. Membrane
potentials were measured with a WP Instruments M4-A electrometer
amplifier (Sarasota, FL), and the output was displayed using a MacLab
System (Castle Hill, Australia). Measurements were made only in cells
(from at least four different preparations) that gave a stable
membrane potential within 10 s of penetration, which indicates a
good seal of the plasma membrane with the recording electrode.
Western Blots—Cultured cells were harvested, as reported
previously (1), and then
washed twice in ice-cold Krebs-Henseleit bicarbonate medium, pH 7.4.
Whole cell extracts were made by freeze-thaw cycles in a buffer
containing 150 mM NaCl, 1 mM
EDTA, 10 mM Tris-HCl, 1 mM
phenylmethylsulfonyl fluoride, 1 µg/ml leupeptin, 1 µg/ml aprotinin,
and 1 µg/ml pepstatin, pH 7.4. Fifty µg of protein (as
determined by the Bradford assay) were boiled with Laemmli buffer and
resolved in 12.5% SDS-PAGE. Proteins were transferred to a
nitrocellulose membrane and subjected to Western blotting with
anti-cystic fibrosis transmembrane conductance regulator (anti-CFTR)
monoclonal antibody (CFTR (CF3) antibody from Novus Biologicals,
Littleton, CO). Immunizing peptide corresponds to amino acid residues
103–1117 found in the first extracellular loop of human CFTR. This
sequence is conserved in mice. Blots were developed using
horseradish peroxidase-conjugated secondary antibody and enhanced
chemiluminescence (ECL system, Amersham Biosciences).
Reverse Transcription-PCR—A 392-bp region corresponding to
nucleotides 1340–1730 of the mouse CFTR gene (22) was
amplified with the forward primer 5'-GGGAGGAGGGATTTGGGGAA-3'
and the reverse primer 5'-GTGATGTCCTGCTGTAGTTG-3'. The CFTR
cDNA was obtained using a random hexamer primer and a MultiScribe
Reverse Transcriptase kit as described by the manufacturer (TaqMan
RT Reagents, Applied Biosystems, Foster City, CA). A PCR master
mix containing the specific primers and AmpliTaq Gold DNA polymerase
(Applied Biosystems) were then added. Amplifications were performed
in a GeneAmp 2400 thermal cycler (PerkinElmer Life Sciences).
Northern Blot Analysis—Total RNA was isolated using the
TRIzol kit from Invitrogen and
following the manufacturer's instructions. Ten µg of total RNA were
electrophoresed in 1% agarose gels containing formaldehyde. RNA was
electrophoretically transferred to a nylon membrane (GeneScreen;
PerkinElmer Life Sciences) and covalently bound to the membrane
following UV cross-linking. Murine CFTR and control
glyceraldehyde-3-phosphate dehydrogenase (G3PDH, Clontech) cDNA
probes were radiolabeled with 32P by random priming (23). The
membranes were hybridized at 65 °C with the 32P-labeled
cDNA fragments. After washing at room temperature, the filters were
exposed to film, and autoradiography was performed using a
PhosphorImager (Bio-Rad).
Perifusion of B16M-F10 Cells—Isolated B16M cells, suspended
in DMEM, were incubated in a perifusion system similar to that
described previously for rat hepatocytes (24). Briefly, a
buffer gassed with 95% O2, 5% CO2 was
constantly pumped by an LKB multiperpex roller pump (type 2115;
Amersham Biosciences) to a chamber containing a final volume of 10 ml
and 3 x 106 B16M-F10 cells per ml.
The filter (Ultracel Amicon YM100 membrane, Millipore,
Billerica, MA) was placed at the top of the chamber. The tumor cell
suspension was perifused at 37 °C and maintained at a homogenous
state by using a magnetic stirrer placed at the bottom of the
chamber. The perifusion buffer was Krebs-Henseleit bicarbonate
medium, pH 7.4, containing plasma concentrations (aortic blood) of
free L-amino acids found in tumor-bearing
C57BL/6J mice (395 ± 34 µM Ala, 56 ± 8 µM Asn, 24 ± 3 µM Asp, 8 ± 1
µM Cyst(e)ine, 72 ± 18 µM
Glu, 442 ± 37 µM Gln, 229 ± 36 µM Gly, 46 ± 11 µM His, 62 ± 13
µM Ile, 78 ± 16 µM Leu, 265
± 23 µM Lys, 44 ± 6 µM Met,
56 ± 9 µM Phe, 107 ± 11 µM
Pro, 87 ± 24 µM Ser, 117 ± 14 µM Thr, 70 ± 15 µM Trp, 83 ± 10 µM Tyr, 115 ± 18 µM Val, 129 ± 16
µM Arg; n = 15), GSH (9 ± 1 µM), glucose (1 g/liter), sodium pyruvate (10
mg/liter), albumin-free fetal calf serum (1.0%, Invitrogen) and
supplemented with 10 units/ml penicillin and 10 µg/ml streptomycin.
Perfusate flow (2 ml/min) was constant throughout the experiment.
Effluent flow was monitored continuously for O2 and pH
with Philips electrodes. Tumor cell viability was always >97%
along the experimental time. A syringe was introduced into the
chamber through a rubber septum to take samples (0.5 ml) of the cell
suspension without interrupting the flow.
Amino Acid Analysis—Proteins were precipitated by treating
0.1 ml of intracellular compartment or plasma with 0.4 ml of
3.75% (w/v) ice-cold sulfosalicylic acid in 0.3 M
lithium citrate buffer, pH 2.8. After centrifugation, 0.25 ml of the
supernatant were injected into an LKB 4151 amino acid analyzer
(Amersham Biosciences) (25).
Verapamil and Acivicin Cellular Pharmacokinetics—Accumulation
of N-methyl[3H]verapamil (VRP) (
-[3-[[2-(3,4-dimethoxyphenyl)ethyl]
methylamino]propyl]-3,4-dimethoxy-
-(1-methylethyl)monohydrochloride) or
[3H]acivicin (ACV) (L-(
S,5S)-
-amino-3-chloro-4-dihydro-5-isoxazole acetic acid)
(PerkinElmer Life Sciences) in perifused cells was measured as
described previously (26, 27). Briefly,
B16M-F10 cells were perifused (see above) in the presence of 1 µM [3H]VRP (0.05 µCi/ml) or 2 µM [3H]ACV (0.1 µCi/ml). Aliquots of
perifused cells (0.2 ml) were taken from the chamber each 10 min, and
accumulation of [3H]VRP or [3H]ACV was stopped
by rapid dilution into ice-cold PBS. Cells were centrifuged and
washed twice with 1 ml of ice-cold PBS. After centrifugation, cell
pellets were solubilized in 1% SDS, and cell-associated radioactivity
was determined by liquid scintillation counting.
GSH-related Enzyme Activities—B16M-F10 cells were detached
(see above), washed twice at 4 °C in Krebs-Henseleit bicarbonate
medium (without Ca2+ or Mg2+) containing 0.5 mM EGTA, pH 7.4, resuspended, and homogenized in 0.1
M phosphate buffer, pH 7.2, at 4 °C.
-Glutamylcysteine synthetase (
-GCS) and GSH synthetase activities were measured as
described elsewhere (4).
-Glutamylcysteine Synthetase Expression Analysis—Total
RNA was isolated by the acid phenol guanidine method (28). cDNA
was obtained using a random hexamer primer and a MultiScribe
reverse transcriptase kit as described by the manufacturer (TaqMan
Reverse Transcription Reagents, Applied Biosystems, Foster City,
CA). A PCR master mix containing the specific primers (
-GCS-heavy subunit (
-GCS-HS): forward, 5'-ATC CTC CAG TTC CTG CAC ATC TAC, and
reverse 5'-GAT CGA AGG ACA CCA ACA TGT ACTC;
-GCS-light subunit (
-GCS-LS): forward, TGG AGT TGC ACA GCT GGA CTC T, and
reverse 5'-CCA GTA AGG CTG TAA ATG CTC CAA; G3PDH: forward,
5'-CCT GGA GAA ACC TGC CAA GTA TG, and reverse 5'-GGT CCT CAG
TGT AGC CCA AGA TG) and AmpliTaq Gold DNA polymerase (Applied
Biosystems) were then added. Real time quantitation of
-GCS-HS and
-GCS-LS mRNA relative to G3PDH mRNA was performed with a
SYBR Green I assay and evaluated using a iCycler detection system
(Bio-Rad). Target cDNAs were amplified in separate tubes using
the following procedure: 10 min at 95 °C and then 40 cycles of
amplification (denaturation at 95 °C for 30 s and annealing and
extension at 60 °C for 1 min per cycle). The increase in fluorescence
was measured in real time during the extension step. The threshold
cycle (CT) was determined, and then the relative
gene expression was expressed as follows: fold change = 2-
(
CT), where
CT = CT target -
CT G3PDH, and
(
CT) =
CT treated -
CT control.
Measurement of H2O2—The assay of
H2O2 production was based on the
H2O2-dependent oxidation of the homovanillic acid
(3-methoxy-4-hydroxyphenylacetic acid) to a highly fluorescent dimer
(2,2'-dihydroxydiphenyl-5,5'-diacetic acid) that is mediated by
horseradish peroxidase (3).
Cystine Uptake—B16M-F10 cells were plated in 25-cm2
culture dishes. At the required times, cells were rinsed three
times with prewarmed transport medium (10 mM
PBS, pH 7.4, with 0.01% CaCl2, 0.01% MgCl2 and
0.1% glucose). Uptake measurement was initiated by addition of 1.0 ml
of transport medium containing 1 µCi of L-[3H]cystine (Amersham Biosciences) and
nonradioactive L-cystine (0.5 mM). After incubation at 37 °C, uptake was finished by
rinsing several times with ice-cold PBS until less than 0.001% of the
initial radioactivity was present in the supernatant. Cells were then
dissolved with 0.5 ml of 0.5 N NaOH, and an
aliquot was used for determining radioactivity and another for
protein assay. To correct for trapping, transport at 4 °C was studied
in parallel (29).
-Glutamyltranspeptidase Assay—
-GT activity was measured as described previously (30). Protein was
determined with the BCA protein assay (Pierce).
Isolation and Culture of Hepatic Sinusoidal Endothelium—Syngenic
male C57BL/6J mice (10–12 weeks old) were from IFFA Credo
(L'Arbreole, France) and received care according to the criteria
outlined by the National Institutes of Health. Hepatic sinusoidal
endothelium (HSE) was separated in a 17.5% (w/v) metrizamide
gradient and identified as described previously (3). Cultures
of HSE were established and maintained in pyrogen-free DMEM
supplemented as described above for the B16M cells. Differential
adhesion of endothelial cells to the collagen matrix and washing
allows a complete elimination of other sinusoidal cell types
(Kupffer, stellate, lymphocytes) from the culture flasks.
B16M-F10-Endothelial Cell Adhesion and Cytotoxicity Assays—
B16M-F10 cells were loaded with
2',7'-bis(2-carboxyethyl)-5,6-carboxyfluorescein acetoxymethyl ester
(BCECF-AM, Molecular Probes) (106 cells were incubated in
1 ml of HEPES buffered DMEM, containing 50 µg of BCECF-AM and 5 µl of
Me2SO, for 20 min at 37 °C). After washing,
BCECF-AM-containing cells were resuspended in HEPES buffered DMEM
without phenol red at a concentration of 2.5 x 106 cells/ml and added (0.2 ml/well) to
endothelial cells (plated 24 h before) and also to plastic or
collagen pre-coated control wells. The plates were then incubated at
37 °C, and 20 min later the wells were washed three times with
fresh medium and read for fluorescence using a Fluoroskan Ascent
FL (Labsystems, Manchester, UK). The number of adhering tumor
cells was quantified by arbitrary fluorescence units based on
the percentage of the initial number of B16M-F10 cells added to
the HSE culture (3). Damage to
B16M-F10 cells during their in vitro adhesion to the HSE was
measured, as described previously (2), using tumor
cells loaded with calcein-AM (Molecular Probes). The integrity of
B16M-F10 cells cultured alone was assessed by trypan blue exclusion
and by measuring lactate dehydrogenase activity released to the
extracellular medium (1). Other
reagents used in experiments of tumor cytotoxicity were from
Sigma.
Cytokines—Recombinant murine TNF-
(2 x 107 units/mg protein) and
recombinant murine (IFN-
; 105 units/mg protein) were obtained from Sigma.
Stock solutions (5 x 105 units
TNF-
/ml and
25 x 104 units of
IFN-
/ml) were diluted in sterile physiological saline solution
(0.9% NaCl), adjusted to pH 7.0, and stored at 4 °C.
In Vivo Microscopy—C57BL/6J mice were fed ad libitum on
a stock laboratory diet (Letica, Barcelona, Spain) and kept on
a 12-h light/12-h dark cycle with the room temperature maintained at
22 °C. Procedures involving animals were in compliance with the
national and international laws and policies (EEC Directive 86/609,
OJ L 358. 1, December 12, 1987; and National Institutes of Health
Guide for the Care and Use of Laboratory Animals Number 85-23). In
vivo microscopy to follow metastatic cell dynamics within the
liver was performed, as earlier described (2), using
calcein-AM (Molecular Probes)-labeled B16M-F10 cells. The total
number of calcein-AM-labeled cells per hepatic lobule was recorded
in 10 different lobules per liver at 15-min intervals for a 6-h
period. The microscope was an Eclipse E600FN, providing
transillumination or epi-illumination, equipped for video microscopy
using a digital DXM 1200 camera (Nikon, Tokyo, Japan).
Apoptosis Detection—The livers used for in vivo microscopy
studies were fixed in formalin embedded in paraffin and then
processed in 10-µm thick sections for routine hematoxylin
staining. Immunohistochemical detection of apoptosis at single
cell level, based on labeling of DNA strand breaks (TUNEL), was
followed using the in situ cell death detection kit POD from
Roche Diagnostics. Sections were examined under a Leica Microsystems
(Nussloch, Germany) upright microscope with standard x10, x40, and
x63 plan apo-objectives.
Experimental Metastases—Hepatic metastases were produced by
intravenous injection (portal vein) into anesthetized mice (nembutal,
50 mg/kg intraperitoneally) of 4 x
105 viable B16M-F10 cells suspended in 0.2 ml of DMEM.
Mice were cervically dislocated 10 days after B16M-F10 inoculation.
The livers were fixed with 10% formaldehyde in PBS (pH 7.4) for 24 h
at 22 °C and then paraffin-embedded. Metastasis density (mean number
of foci/100-mm3 of liver detected in 15 10 x 10-mm2 sections per liver) and
metastasis volume (mean % of liver volume occupied by metastasis)
were determined as described earlier (1).
Expression of Results and Statistical Significance—Data
were analyzed by one- or two-way ANOVA or t tests where
appropriate (SPSS 9.0 software for Windows; SPSS Inc., Chicago). The
homogeneity of the variances was analyzed by the Levene test. The
null hypothesis was accepted for all the values of the tests in which
the F value was nonsignificant at p > 0.05. The data
for which the F value was significant was examined by Tukey's
test at p < 0.05.
 |
RESULTS |
MRP1
and a BCL-2-dependent System Channel GSH Efflux from B16M-F10
Cells—Recently, we reported that GSH efflux was significantly
reduced in BCL-2-overexpressing B16M-F10 cells (5).
Moreover, GSH release increased in B16M-F10/Tet-BCL-2 cells loaded
with anti-BCL-2 antibodies (5), thus
suggesting that BCL-2 directly inhibits the transport system.
We investigated further the mechanisms through which GSH is
released from highly metastatic cells. As shown in Table I,
GSH efflux from B16M-F10 cells was decreased by L-methionine suggesting that, in part, GSH is released
through a system possibly similar to an L-methionine-sensitive sinusoidal GSH transporter
detected in hepatocytes (31, 32). However,
GSH efflux was not inhibited by L-methionine in
B16M-F10/Tet-BCL-2 (Table I),
suggesting that likely BCL-2 and L-methionine
act on the same transport system (similar results were reported
previously in HeLa cells (33)). Further
experiments using MRP-/-1 or MRP-/-2 clones showed that GSH efflux
was decreased, as compared with controls, in MRP-/-1 B16M-F10 and in
B16M-F10/Tet-BCL-2 cells (Table I).
GSH efflux in MRP-/-1 B16M-F10/Tet-BCL-2 cells was practically
abolished, and identical results were obtained in MRP-/-1 B16M-F10
cells incubated in the presence of methionine (Table I).
Taken together these results indicate that a BCL-2-dependent
system and MRP1 are the main mechanisms channeling GSH efflux
from B16M-F10 cells.
View this
table: [in this
window] [in a new window] |
TABLE I BCL-2-
and MRP-dependent GSH efflux from B16M-F10 cells B16M-F10 cells
were cultured for 24 h. Some flasks were used to measure rates of
GSH efflux (to prevent degradation of the GSH accumulated in the
extracellular space the -glutamyltranspeptidase was blocked by adding 10 µM ACV to the culture medium 3 h before measuring
efflux; see Ref. 4).
Other flasks were used for GSH, GSSG, and BCL-2 determination. GSH
efflux corresponded practically to GSH since GSSG was, in all
conditions, 1–3% of the total glutathione found in the extracellular
space (not shown). BCL-2 levels in control and Tet-BCL-2-treated
B16M-F10 cells were 27 ± 5 and 118 ± 20 units/mg protein,
respectively (n = 5 in each case, p < 0.01).
Addition of L-methionine (1 mM) or the use of MRP-/-1 or MRP-/-2 clones did not
alter significantly the BCL-2 levels (not shown). A one-way ANOVA
was performed for comparison among groups. Different superscript
letters within a column indicate differences, p < 0.01.
Results are means ± S.D. of 5–6 independent experiments.
| |
Molecular
Nature of the BCL-2-dependent GSH Channel in B16M-F10 Cells—BCL-2
overexpression has been associated with plasma membrane
hyperpolarization (34), and it was
suggested that changes in the rate of GSH efflux could be the
consequence of changes in membrane polarity (e.g. Ref. 35). We explore
first this possibility in our experimental model. The PMP measured in
B16M-F10, B16M-F10/Tet-BCL-2, and Bcl-2-AS-treated B16M-F10
cells cultured for 24 h was 62.3 ± 7.1, 75.4 ± 6.2 (p <
0.05), and 60.3 ± 5.5 mV, respectively (n = 3–4 in each case).
Therefore, BCL-2 overexpression (B16M-F10/Tet-BCL-2) indeed increased
significantly the PMP as compared with controls (B16M-F10), which in
our model associated with a decrease in GSH efflux (Table I).
However, it has been claimed that an increase in GSH efflux is the
consequence of hyperpolarization (36). However, we
observed that BCL-2 depletion increased GSH efflux from B16M-F10
cells (Table
II) without affecting the PMP (see above), and addition to
cultured B16M-F10 cells of ouabain (1 mM), a
classical inhibitor of the plasma membrane
Na+/K+-ATPase, decreased the PMP to
63% of
control values (see above) but did not affect significantly rates
of GSH efflux measured in controls, in the presence of L-methionine (1 mM) or in MRP-/-1
cells (see e.g. Table I).
Apparently, efflux of GSH is not driven in B16M-F10 cells by a
significant change in the PMP. Our results appear in agreement with
those reported by Liu et al. (37) for Hep G2
cells, a human-derived hepatoma cell line, where a fall in the
membrane potential produced by the replacement of Na+ with
equivalent K+ did not affect GSH efflux significantly.
Neither ouabain, vanadate (a Ca2+-ATPase inhibitor), nor
BaCl2 (a K+ channel blocker) significantly affected
the GSH efflux; however, L-methionine (1 mM) decreased GSH efflux from Hep G2 cells (37).
View this
table: [in this
window] [in a new window] |
TABLE II GSH
efflux and content in perifused B16M-F10 cells treated with Bcl-2-AS
and VRP B16M-F10 cells were cultured for 3 days in the presence
or in the absence of 50 µM Bcl-2-AS or a
2-base mismatch oligodeoxynucleotide (see under "Experimental
Procedures"). Then the cells were harvested, cultured again for 24 h
in the absence of oligodeoxynucleotides, and used for perifusion
experiments. BCL-2 levels in control (none under the heading
Additions), Bcl-2-AS-, or Bcl-2-mismatch-AS-treated
cells were 36 ± 8, 33 ± 6, and 2 ± 1 units/mg protein, respectively
(n = 6–7 in each case). Results obtained by substituting
Bcl-2-AS by its 2-base mismatch counterpart were not
significantly different from those obtained under no additions or in
the presence of VRP alone (not shown). To measure rates of GSH
efflux, 2-ml aliquots of effluent buffer were collected each min for
30 min, starting at the indicated perifusion times, and placed on
ice. One ml was used to measure total glutathione, whereas the other
milliliter was mixed with 1 ml of ice-cold perchloric acid (12%)
containing 40 mM N-ethylmaleimide and 2
mM bathophenanthroline disulfonic acid to
prevent GSH oxidation and to measure GSSG accurately (4)
(see under "Experimental Procedures"). GSH = 2 x (total glutathione - GSSG). Before
determinations were performed, samples were concentrated 40 times by using speed vacuum centrifugation at 4 °C. GSSG
present in the effluent was, in all conditions, 1–2% of the total glutathione. VRP concentration in the
perfusate flow was 1 µM (see Fig.
3 and the text for details regarding its cellular
pharmacokinetics). VRP was added to the perfusate flow as indicated
in the legend to Fig.
3. No significant differences were found when results obtained
with control and cells preincubated with the 2-base mismatch
oligodeoxynucleotide were compared (not shown). Data are means ±
S.D. for 6–7 independent experiments. A two-way ANOVA was used to
make comparisons among different treatments and time points.
Perifusion time is given in hours. Different superscript letters
indicate differences, p < 0.05.
| |
The
CFTR, a member of the ABC family of membrane transport proteins with
structural similarities with the MRP1, forms a phosphorylation- and
ATP-dependent channel permeable to Cl- and to other larger
organic anions, including GSH (38). The CFTR is
expressed in different cell types (39), and thus we
also explored this possibility. GSH efflux increased significantly in
Bcl-2-AS-treated B16M-F10 cells (containing
3% of
control BCL-2 levels, see the legend to Fig. 1), whereas
GSH efflux decreased to
50% of
control values in MRP-/-1 B16M-F10 cells (Fig. 1). The
difference represents the BCL-2-dependent GSH efflux (see also data
in Table I).
Monoclonal antibodies anti-CFTR practically abolished the
BCL-2-dependent GSH efflux from B16M-F10 cells (Fig. 1; the
presence of CFTR in B16M-F10 cells was confirmed with a Western blot,
not shown). In consequence, addition of anti-CFTR antibodies to
Bcl-2-AS-treated MRP-/-1 B16M-F10 cells practically abolished
GSH efflux (Fig.
1).
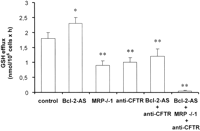 View larger
version (16K): [in this
window] [in a new window] |
FIG. 1. Effect
of monoclonal antibodies anti-CFTR on the GSH release from B16M-F10
cells. B16M-F10 cells were cultured for 24 h. BCL-2 levels in
control and in Bcl-2-AS-treated cells were 30 ± 6 and 1 ± 0.5
units/mg protein, respectively (n = 4–5 in each case,
p < 0.01). BCL-2 levels were not affected significantly in
MRP-/-1 cells or by the addition of anti-CFTR antibodies (not
shown). Results obtained by substituting Bcl-2-AS (in
Bcl-2-AS- or Bcl-2-AS + anti-CFTR-treated cells) by
its 2-base mismatch counterpart were not significantly different
from those obtained in controls or in the presence of anti-CFTR
alone (not shown). Data are means ± S.D. for 4–5 different
experiments. The significance test (Student's t test) refers
to the comparison of each group versus control values
(*, p < 0.05; **, p <
0.01).
| |
CFTR
is activated by the binding of ATP to its cytoplasmic nucleotide-binding
domain and by phosphorylation of key serine residues in the
regulatory domain. Phosphorylation is mediated principally by
cAMP-dependent protein kinase A (PKA) and by protein kinase C
(although to a less degree than the activation by PKA) (40).
Basal expression of the CFTR gene is dependent on PKA activity
(41).
Treatment of human colon carcinoma T84 cells with the PKA-selective
inhibitor
N-[2-(p-bromocinnamylamine)ethyl]-5-isoquinolinesulfonamide
(H-89) (Seikagaku America, Rockville, MD) caused a complete
suppression of CFTR gene expression without affecting other
constitutively active genes (41). Thus, we
also used this approach in our experimental model. As shown in Fig. 2 treatment
with H-89 inhibited CFTR expression in B16M-F10 and in MRP-/-1
B16M-F10 cells. For the conditions used in Fig. 2, rates of
GSH efflux (nmol/106 cells x h) from wild-type B16M-F10 cells were 1.7
± 0.2 (similar to control values in Fig. 1); from
MRP-/-1 B16M-F10 cells were 0.8 ± 0.2 (similar to its equivalent
value in Fig. 1); from
H-89-treated B16M-F10 cells were 1.0 ± 0.15 (similar to the value
found in the presence of anti-CFTR, Fig. 1); and from
H-89-treated MRP-/-1 B16M-F10 cells were 0.04 ± 0.02 (which
corresponds approximately to a 98% decrease in the rate of efflux)
(n = 4 for each condition; and p < 0.01 in all
cases, as compared with control wild-type B16M-F10 cells).
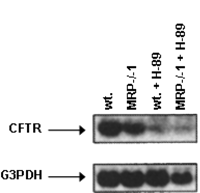 View larger
version (40K): [in this
window] [in a new window] |
FIG. 2. Absence
of CFTR mRNA in B16M-F10 and MRP-/1 B16M-F10 cells treated with a
PKA-selective inhibitor. Northern blot of total cellular RNA (10
µg). From left to right: 1st lane, wild-type
(wt) B16M-F10 cells; 2nd lane, MRP-/-1 B16M-F10 cells
(MRP-/-1); 3rd lane, B16M-F10 cells
treated with H-89 (wt + H-89); 4th lane
(MRP-/-1 + H-89). H-89 (30 µM; Ref. 41)
was added to 24-h cultured cells. Total RNA was isolated 24 h later.
| |
These
results indicate that CFTR is directly involved in channeling GSH
from the cytoplasm of B16M-F10 cells to the extracellular space and
that this mechanism corresponds to the BCL-2-sensitive channel.
Nevertheless we cannot rule out the possibility that other
mechanism(s) could also be working in other cell types or that
different CFTR gene mutations could be found when comparing different
cancer cells. These interesting questions, although far beyond the
aim of the present report, deserve further investigation.
Antisense Bcl-2 Oligodeoxynucleotides and Verapamil Accelerate
GSH Release from B16M-F10 Cells—Parallel to the BCL-2-sensitive
CFTR, two mechanisms of transport of GSH by MRP1 have been suggested
as follows: passive permeability and a VRP-dependent active
transport (42). VRP, an
inhibitor of P-glycoprotein-mediated drug efflux, is not transported
by MRP1 (43)
but may also inhibit MRP1-mediated drug extrusion (44). We tested
VRP in combination with Bcl-2-AS treatment to potentiate GSH
efflux from B16M-F10 cells. A perifusion chamber, containing a
suspension of B16M-F10 cells, was used as an experimental setup that
mimics in vivo conditions by providing a constant supply of
glucose, amino acids, and GSH at physiological plasma concentrations
(see under "Experimental Procedures"). This setup allowed us to use a
VRP concentration (1 µM) that corresponds to
clinically accepted and nontoxic levels in plasma (45)
(dose-response studies showed that 0.5–2 µM VRP
activates GSH efflux from perifused cells in a
concentration-dependent fashion, data not shown). VRP accumulation
within perifused B16M-F10 cells peaked 10–20 min (
70
pmol/106 cells) after addition to the perfusate flow and
then decreased reaching a lower steady-state concentration at 60 min
(
40 pmol/106 cells) (Fig. 3). These
values of VRP accumulation, which are in agreement with those
reported previously in HeLa cells (43), were not
changed significantly when control and BCL-2-AS-treated cells were
compared (Fig.
3).
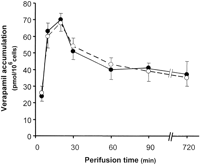 View larger
version (17K): [in this
window] [in a new window] |
FIG. 3. Time
course of [3H]verapamil uptake by perifused B16M-F10
cells. [3H]VRP accumulation by control (•) or
Bcl-2-AStreated ( ) cells was determined over 720 min of perifusion time (see
under "Experimental Procedures"). The concentration of VRP (1 µM in the perfusate flow) was maintained constant
during the perifusion. Data points are means ± S.D. of 4–5
independent determinations.
| |
As
shown in Table
II, Bcl-2-AS and VRP independently increased rates of GSH
efflux from perifused B16M-F10 cells. In fact, VRP had no effect on
GSH efflux in MRP-/-1 B16M-F10 cells (not shown), and when
Bcl-2-AS and VRP were both present their effects were additive
and constant along a 12-h perifusion time (GSH efflux was increased
by
100%, Table II).
Intracellular GSH contents were significantly decreased (
30%), as
compared with controls, in Bcl-2-AS- and VRP-treated B16M-F10
cells after 6 h of perifusion (Table III).
However, at 12 h of perifusion time, GSH levels were
70% higher
in Bcl-2-AS- and VRP-treated B16M-F10 cells than in controls
(Table II).
Thus, it appeared plausible that loss of GSH accelerates their rate
of intracellular synthesis.
View this
table: [in this
window] [in a new window] |
TABLE III GSH
synthesis in perifused B16M-F10 cells under conditions of Bcl-2-AS-
and/or VRP-induced acceleration of GSH release B16M-F10
cells were cultured and perifused as indicated in the legend to Table
II. For GSH synthesis, cells were taken from the perifusion
chamber, washed twice, and resuspended in ice-cold Krebs-Henseleit
bicarbonate medium, pH 7.4, and incubated (5 mg of dry weight/ml)
(1),
in 10-ml-Erlenmeyer flasks (final volume 2 ml) for 60 min, at 37 °C,
in the presence of the amino acid precursors for GSH synthesis (5
mM L-Gln, 2 mM Gly, 1 mM L-Ser, 1
mM N-acetylcysteine). Glucose (5 mM) and bovine serum albumin (2%) were always present.
GSH synthesis was calculated from total GSH content at 0, 20, 40,
and 60 min of incubation. No significant differences were found when
data displayed in the table for 6 h were compared with those
obtained at 1 h of perifusion time (not shown). GSH-S activity in
controls (see None under the heading Additions) was 15 ± 3
milliunits/106 cells (this value did not change
significantly along the perifusion time even in the presence of
Bcl-2-AS- and/or VRP, n = 4–5 in each case, not
shown). Results obtained by substituting Bcl-2-AS by its
2-base mismatch counterpart were not significantly different from
those obtained under no additions or in the presence of VRP alone
(not shown). Data are means ± S.D. for 8–10 independent experiments.
Perifusion time is given in hours. A two-way ANOVA was used to make
comparisons among groups. Different superscript letters indicate
differences, p < 0.01.
| |
Antisense
Bcl-2 Oligodeoxynucleotides- and/or Verapamil-induced
Acceleration of GSH Efflux Associates with
-Glutamylcysteine Synthetase Overexpression in B16M-F10
Cells—As shown in Table III, either
both or Bcl-2-AS or VRP treatment significantly increased the
rate of GSH synthesis in B16M-F10 cells. Moreover, their effects
appeared additive and associated with an increase in
-GCS activity (Table III).
Bcl-2-AS and/or VRP, as compared with controls, did not change
significantly the GSH synthetase activity (see Table III
legend). Moreover, as shown in Table IV,
the increase in
-GCS activity was accompanied by a previous increase in both
-GCS-HS and
-GCS-LS expression (maximum values were found at 3 h).
Therefore, increased GSH efflux associates with
-GCS overexpression.
Changes
in
-GCS activity can result from transcriptional and
post-transcriptional regulation affecting the HS and/or the LS
(for review see Ref. 46).
Intracellular GSH depletion, e.g. that induced by
GSH-conjugating agents such as diethyl maleate, increased
transcription of both subunits (46) as it occurs
in our experimental conditions. Moreover, oxidative stress,
which may arise as a consequence of GSH depletion and increased
intracellular levels of H2O2 and OH·, can also
induce increased transcription of both subunits (46). In fact,
Bcl-2-AS- and VRP-induced GSH depletion (Table II) is
accompanied by an increase in H2O2 production
by the B16M-F10 cells (13 ± 4 in controls and 25 ± 5 nmol of
H2O2/106cells x h in Bcl-2-AS- and VRP-treated cells,
n = 5, p < 0.01).
Inhibition of
-Glutamyltranspeptidase Activity Prevents GSH Content
Increase in B16M-F10 Cells Treated with Antisense Bcl-2
Oligodeoxynucleotide and Verapamil—In rapidly growing
tumors cyst(e)ine, whose concentration in blood is low, may
become limiting for GSH synthesis and cell growth (4, 47). Thus,
in order to increase the rate of GSH synthesis, malignant cells
may require alternative pathways to ensure cyst(e)ine availability.
Keeping the extracellular supply of amino acids constant and
physiological during the perifusion (see under "Experimental
Procedures"), the intracellular availability of amino acid precursors
for GSH synthesis was investigated. The concentrations of free
L-glutamate and glycine within the B16M-F10 cells
were constant through the perifusion time (e.g. 3.3 ± 0.4 and
2.5 ± 0.3 mM, respectively, in controls;
n = 6; enough to ensure maximum rates of GSH synthesis (12)) and were
not changed by addition of Bcl-2-AS and/or VRP (not shown).
However, free intracellular L-glutamine and
L-cyst(e)ine were undetectable, which is not
surprising because L-glutamine is a major fuel used
by cancer cells (48), and L-cyst(e)ine is rapidly used for protein and GSH
synthesis (25). L-Cystine is predominant outside the cell because
L-cysteine rapidly autoxidizes to L-cystine in the extracellular fluids, but once it
enters the cell through the Xc- system L-cystine is reduced to L-cysteine (Ref. 46 and
references therein). Thus, we measured L-cystine
uptake by B16M-F10 cells and found that Bcl-2-AS and/or VRP
did not significantly affect this rate (e.g. 0.41 ± 0.08
nmol/mg protein in controls, n = 5). Nevertheless, we showed
that tumor
-GT activity and an intertissue flow of GSH increase GSH
content in B16M-F10 cells and work as a tumor growth-promoting
mechanism (4).
-GT cleaves extracellular GSH-releasing
-glutamyl-amino acids and cysteinylglycine, which is further
cleaved by membrane-bound dipeptidases into L-cysteine and glycine (12). Free
-glutamyl amino acids, L-cysteine and glycine,
entering the cell serve as GSH precursors (12). Hence,
-GT expression may provide tumor cells with a growth
advantage at physiological concentrations of L-cyst(e)ine (4, 47). Therefore,
if the increase in GSH content (Table II)
following the effect of Bcl-2-AS and VRP on GSH efflux depends
on L-cyst(e)ine availability, and if this is
provided in part by the
-GT, then inhibition of this activity could limit GSH
synthesis. We tested this possibility by adding to the perifusion
system ACV, an irreversible
-GT inhibitor (49). ACV was
present in the perfusate flow for only 30 min (Fig. 4). ACV
accumulation within the B16M-F10 cells peaked 10–20 min (
20
pmol/106 cells) after addition, and then its concentration
decreased to a steady-state low level (
4
pmol/106 cells) (Fig. 4; these
values were not changed when control and Bcl-2-AS- or
VRP-treated cells were compared, data not shown). ACV decreased
-GT activity to nondetectable levels and decreased GSH
synthesis but did not affect the rate of GSH efflux (Table V) or the
rate of L-cystine uptake (not shown, see above
for control values). However, the rate of GSH synthesis in B16M-F10
cells treated with Bcl-2-AS, VRP, and ACV was found similar to
controls (as under "no additions" in Table V)
when the concentration of L-cysteine in the
perifusion buffer was increased 2-fold (up to 16 µM). Hence, intracellular L-cysteine
availability indeed appears modulated by its
-GT-dependent generation from extracellular GSH. Therefore,
we conclude that Bcl-2-AS- and VRP-induced acceleration of GSH
efflux combined with inhibition of
-GT promote GSH depletion in B16M-F10 cells.
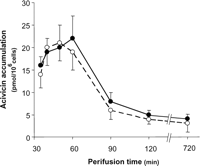 View larger
version (16K): [in this
window] [in a new window] |
FIG. 4. Time
course of [3H]acivicin uptake by perifused B16M-F10
cells. [3H]ACV accumulation by control (•)or
Bcl-2-AS-treated ( ) cells was determined over 720 min of perifusion time (see
under "Experimental Procedures"). [3H]ACV (1 µM) was added to the perfusate flow at 30 min of
perifusion and was present for 30 min. Data points are means ± S.D.
of 4–5 independent determinations.
| |
View this
table: [in this
window] [in a new window] |
TABLE V Effect
of ACV-induced inhibition of -GT on GSH content in perifused B16M-F10 cells treated
with Bcl-2-AS and VRP B16M-F10 cells were cultured and perifused
as indicated in the legend to Table
II. VRP addition and concentration in the perfusate flow are
shown in Table
II and Fig.
3. ACV concentration in the perfusate flow was 1 µM (see Fig.
4 and the text for details regarding its cellular
pharmacokinetics). ACV was added to the perfusate flow as indicated
in the legend to Fig.
4. GSH content and -GT activity were measured at 12 h of perifusion time. GSH
synthesis and efflux were measured, starting at 12 h of perifusion,
as indicated in the legend to Table
III but using a complete mixture of amino acids as precursors.
This mixture contained plasma concentrations (aortic blood from
non-tumor-bearing C57BL/6J mice) x
10 of GSH and free L-amino acids (see under
"Perifusion of B16M-F10 Cells" under "Experimental Procedures").
Results obtained by substituting Bcl-2-AS by its 2-base
mismatch counterpart were not significantly different from those
obtained in the presence of VRP or VRP + ACV (not shown). Data are
means ± S.D. for 5–6 different experiments. A one-way ANOVA was
performed for comparison among groups. Different superscript letters
within a column indicate differences, p < 0.01.
| |
Sensitizing
of B16M-F10 Cells to Vascular Endothelium-induced Tumor
Cytotoxicity—Previously, we demonstrated that B16M-F10 cells with
low GSH content (cultured in the presence of BSO), as compared with
untreated controls, were more sensitive to HSE-induced metastatic
cell cytotoxicity (2). However BSO,
when administered under in vivo conditions, decreases GSH in
tumor and non-tumor tissues thus placing the normal tissues at a
disadvantage (6). Thus, we
investigated whether a combination of Bcl-2-AS, VRP, and ACV
could be as effective as BSO but without its nonselective effect on
tissue GSH. First, we assayed the effect of BSO versus
Bcl-2-AS + VRP + ACV on the in vitro interaction between
B16M-F10 and HSE cells. As shown in Table VI, both
treatments decrease tumor GSH to 35–40% of control values. However,
HSE-induced tumor cell death was much higher in the presence of
Bcl-2-AS + VRP + ACV (
96%) than
in the presence of BSO (
66%) (Table VI).
This is in agreement with our previous report (5) showing
that depletion of GSH and BCL-2 levels practically avoids
B16M-F10 survival during interaction with the vascular endothelium.
Also in agreement with this previous report (5), GSH depletion
did not alter the % of tumor cell adhesion to the HSE. To prove
that GSH is directly regulating metastatic cell survival, we
tested the effect of GSH ester (which enters the cell and delivers
free GSH (4)). As shown in
Table VI, GSH
ester prevented HSE-induced B16M-F10 cytotoxicity in the presence of
BSO, but only partially in the presence of Bcl-2-AS, VRP, and
ACV (where BCL-2 depletion remains, see Ref. 5). Second, we
assayed the effect of Bcl-2-AS, VRP, and ACV on the in
vivo B16M-F10 cell arrest and viability within the hepatic
microvasculature. Calcein-labeled B16M-F10 cells, which present a
green fluorescent cytoplasm, arrested in the liver sinusoids after
intraportal inoculation (Table VII).
The number of arrested B16M-F10 and Bcl-2-AS-treated B16M-F10
cells was similar (Table VII) and
constant along the time (no significant differences were found within
the 30–360-min postinjection) (not shown). As reported previously (2), the
number of arrested cells was not altered by depleting tumor
cell GSH or by treating mice with lipopolysaccharide (LPS) (to
pre-activate the endothelium) before tumor cell inoculation (Table VII). In
physiological saline- or VRP + ACV-treated mice (Table VII),
almost all arrested non-Bcl-2-AS-pretreated B16M-F10 cells
appeared as round bright fluorescent cells of well delineated profile
(nondamaged "intact" cells because no fluorescence diffusion from
their cytoplasm to their neighboring tissue was observed).
Bcl-2-AS pretreatment decreased the viability of arrested
metastatic cells (Table VII), which
appeared as irregularly shaped fluorescent cells with a spread of
diffuse fluorescence staining the contiguous hepatic tissue, and were
considered as damaged with cytoplasmic leakage. The decrease in
B16M-F10 viability was sharp in LPS + VRP + ACV-treated mice
inoculated with Bcl-2-AS-pretreated B16M-F10 cells (only 10%
of all arrested cells remained intact) (Table VII).
Nevertheless, it is important to remark that some apparently intact
metastatic cells may have already activated apoptotic mechanisms (see
Fig. 5).
View this
table: [in this
window] [in a new window] |
TABLE VI Effect
of Bcl-2-AS-, VRP-, and ACV-induced tumor GSH depletion on the in
vitro interaction between B16M-F10 cells and the vascular
endothelium B16M-F10 cells were previously cultured for 3 days
in the presence or in the absence of 50 µM
Bcl-2-AS as indicated in the legend to Table
III. The cells were then harvested and cultured again in the
absence of oligodeoxynucleotides. Twenty four-hour cultured HSE
cells ( 2.5 x 105
cells/well) were co-cultured with B16M-F10 cells ( 5.0 x 105
cells/well; precultured for 24 h). Twenty minutes after B16M-F10
addition to the HSE, the plates were washed as described under
"Experimental Procedures." The ratio of tumor cells adhering to the
HSE was 1:1. TNF- (100 units/ml) and IFN- (50 units/ml), used as a potent activators of NO and
H2O2 generation by the HSE (3),
were added to the co-cultures when all tumor cells present were
attached to the HSE. In endothelium-induced B16M-F10 cytotoxicity
assays, tumor cytotoxicity (expressed as the % of tumor cells that
lost viability within the 4–6-h period of incubation, see
"Experimental Procedures") was determined after 6 h of incubation.
During the 6-h period of incubation, the percentage of HSE cell
viability was 98–99% in all cases. When adding TNF- (100 units/ml) and IFN- (50 units/ml) to cultured B16M-F10 cells alone, no
cytostatic or cytotoxic effects were observed within the next 6 h.
Where indicated B16M-F10 cells were incubated for 24 h with GSH
ester (1 mM), BSO (0.2 mM), VRP (1 µM), or ACV (1 µM) before co-culture with endothelial cells.
Pretreatment of B16M-F10 cells with Bcl-2-AS, VRP, or ACV
individually did not affect significantly control values of tumor
cell adhesion. Pretreatment of B16M-F10 cells with Bcl-2-AS
alone increased tumor cell cytoxicity to 36%, either in the presence or in the absence of GSH ester.
Compared with controls, pretreatment of B16M-F10 cells with VRP or
ACV did not affect significantly the % of tumor cytotoxicity. BCL-2
levels in Bcl-2-AS-treated cells were always <4 units/mg
protein. Data are means ± S.D. for 5–6 independent experiments. A
two-way ANOVA was used to make comparisons among different
treatments. Different superscript letters indicate differences,
p < 0.01.
| |
View this
table: [in this
window] [in a new window] |
TABLE
VII Effect of Bcl-2-AS, VRP, and ACV on the in vivo time
course of B16M-F10 cell arrest and viability within the hepatic
microvasculature Number of arrested cells displayed in the table
were calculated 60 min postinjection (no significant differences
were found when measurements were performed at 30, 120, 180, 240, or
360 min postinjection, not shown). The number of intact cells was
calculated at 6 h postinjection. LPS-treated mice received a single
intravenous injection of 0.5 mg/kg 6 h before B16M-F10 inoculation
(5).
VRP and ACV were administered together using a 2001D ALZET osmotic
pump (Durect Corporation, Cupertino, CA) fixed to a permanent
intravenous catheter. This procedure allowed us to keep plasma
concentrations of VRP and ACV of 1–2 µM
throughout the experimental time. Intravenous administration of VRP
and ACV began 30 min before tumor cell inoculation. In vivo
treatment with VRP or ACV alone did not affect control values of
intact B16M-F10 cells (% arrested in the liver sinusoids). Before
inoculation into mice B16M cells were cultured in the presence or in
the absence of 50 µM Bcl-2-AS as
indicated in the legend to Table
II. GSH contents in control and Bcl-2-AS-treated B16M-F10
cells before inoculation were similar to those reported in Table
II. Results obtained in the presence of the 2-base Bcl-2
mismatch oligodeoxynucleotide were not significantly different from
those obtained in the absence of Bcl-2-AS (not shown). Data
are means ± S.D. for 4–5 different experiments. The significant test
(Student's unpaired t test) refers, for all groups, to the
comparison in the absence or in the presence of Bcl-2-AS
(**, p < 0.01), and also to the difference
between LPS-treated mice and untreated mice (+, p
< 0.05; ++, p < 0.01).
| |
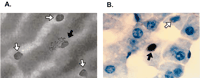 View larger
version (54K): [in this
window] [in a new window] |
FIG. 5. In
vivo microscopic observation of B16M-F10 cell arrest and
apoptotic B16M-F10 cell detection in the liver sinusoids.
A, intravital observation, captured as the negative black and
white picture, of intact (white arrows) and damaged (black
arrow) B16M-F10 cells within the liver sinusoids (3 h after
intrasplenic inoculation). Bar, 25 µm. B, TUNEL
staining showing B16M-F10 cells with intact (white arrow) and
apoptotic (black arrow) nuclei within a liver sinusoid
(diaminobenzidine immunostaining with hematoxylin counterstain,
x63 objective magnification), 3 h
after intrasplenic inoculation. S100 monoclonal antibodies (72)
were used in parallel preparations as control markers of melanocytic
tumor cells within the microvasculature of the hepatic parenchyma
(not shown).
| |
These
in vivo results are in agreement with the in vitro
observations displayed in Table VI and
indicate that GSH and BCL-2 depletion may be a feasible approach to
sensitize metastatic melanoma cells to endothelium-induced
cytotoxicity in vivo. Furthermore, we assayed GSH levels in
different tissues (brain, lung, heart, liver, kidney, pancreas,
skeletal muscle, bone marrow, testis, and erythrocytes, n = 6
in each case) of untreated non-tumor-bearing mice and in LPS-, VRP-
and ACV-treated mice inoculated with Bcl-2-AS-pretreated
B16M-F10 cells (as in the legend to Table VII),
and we found no significant differences as compared with data
reported previously for non-tumor-bearing mice and B16M-F10-bearing
mice (4)
(data not shown), thus indicating that the proposed treatment does
not affect GSH levels in normal tissues.
 |
DISCUSSION |
Analysis
of a Bcl-2 family of genes revealed that B16M-F10 cells (high
metastatic potential), as compared with B16M-F1 cells (low metastatic
potential), preferentially overexpressed Bcl-2 (5). BCL-2
overexpression, without changing the rate of GSH synthesis, induces a
decrease in GSH efflux and, consequently, an increase of GSH content
within B16M-F10 cells (5). Most
B16M cells with a high GSH content survive the NO- and
H2O2-mediated tumoricidal activity of
endothelial cells (50). However,
survival of B16M-F10 during interaction with the vascular
endothelium can be challenged by inhibiting their BCL-2 and GSH
synthesis in vitro (5). Bcl-2
antisense therapy using G3139, for example, an 18-base
phosphorothioate oligonucleotide complementary to the first six
codons of the Bcl-2 mRNA, selectively and specifically
inhibits BCL-2 expression and promotes apoptosis in different
human and murine cancer cell lines (51). Systemic
administration of G3139 to Shionogi tumor-bearing mice led to a rapid
decrease of tumor size (higher when chemotherapy was simultaneously
administered), whereas the oligonucleotide did not affect BCL-2
expression in normal organs (20, 52).
G3139-induced tumor regression without dose-limiting toxicity was
also observed in other tumors, melanoma, lymphoma, or gastric cancers
for example (51).
Furthermore, synergism of the G3139 and anticancer drugs was also
shown in different tumors (53–55). However, on
the other hand, an effective strategy to deplete GSH in metastatic
cells has remained elusive.
B16M-F10 cells surviving the combined nitrosative/oxidative attack
induced by the HSE showed a decrease in GSH content (50).
However, endothelial NO-mediated partial inactivation of
-GCS activity is followed by overexpression of
-GCS-heavy and -light subunits, which leads to a rapid
recovery of GSH levels within invasive cells (50). Therefore,
as suggested by previous achievements in nonmetastatic models (6), a methodology
capable of maintaining low GSH levels in metastatic cells could
represent a critical advance in cancer therapy.
Here we present evidence showing that GSH is released from highly
metastatic B16M-F10 cells through MRP1 and CFTR (Table I and
Fig. 1).
By using a perifusion system that mimics in vivo conditions,
we show that GSH efflux from B16M-F10 cells can be accelerated
be using Bcl-2-AS (which prevents BCL-2-induced inhibition of
GSH release through CFTR) and VRP (which activates GSH release
through MRP1 (42)) (Tables I
and II).
However, Bcl-2-AS and/or VRP treatment associated with
overexpression of
-GCS (Table
IV) increased rates of GSH synthesis
(Table
III) and higher GSH content (Table II) within the B16M-F10 cells.
Recently, we showed that tumor
-GT activity, by providing an extra supply of L-cysteine from extracellular GSH, supports GSH
synthesis in B16M-F10 cells and promotes their metastatic growth (4).
Hence, we tested whether inhibition of this activity could prevent
the increase of GSH content within BCL-2-AS- and VRP-treated B16M-F10
cells. Indeed, as shown in Table III, ACV limited GSH synthesis
and allowed GSH efflux to deplete tumor cell GSH. This strategy,
which combines induction of BCL-2 and GSH depletion, sensitized
perifused B16M-F10 cells to the cytotoxic effects of the vascular
endothelium (Tables VI
and VII).
Can possible clinical applications be derived from our study? The
proto-oncogene Bcl-2 and its anti-apoptotic homologs are
mitochondrial membrane permeabilization inhibitors (56)
and participate in the development of chemoresistance (57),
whereas expression of pro-death genes, e.g. Bax or Bak,
is often reduced in cancer cells (58).
In agreement with this idea, Takaoka et al. (59)
observed that Bcl-2 overexpression in B16M cells enhanced
pulmonary metastasis. In fact, a major form of multidrug resistance
in human tumors is caused by overexpression of the MRP1 gene
(7).
In vivo Bcl-2-AS therapy, as explained above, is feasible. In
addition, VRP (at doses that promote a similar plasma concentration
than that used in our experiments) has already been used in
cancer patients with myeloma or acute lymphocytic leukemia, for
example, where it increased accumulation of daunorubicin or
vincristine within the tumor cells (60).
ACV, the L-glutamine analog anti-metabolite, has
followed phase I and II clinical trials in different tumors (61).
Although its use is limited by severe central nervous system
toxicity, a maximum tolerated dose of 50 mg of
acivicin/m2/day has been proposed in combination with the
amino acid solution aminosyn (which decreases drug uptake in the
central nervous system) (61).
In our studies, ACV concentration in the perfusate flow was 1 µM (present only during 30 min) (Fig. 4 and Table III). By taking into account the
circulating blood volume and the in vivo pharmacokinetics in
humans (61),
this means that ACV doses required to block tumor
-GT activity will remain within nontoxic levels. This is
important because an increased expression of
-GT has been found in melanoma as well as in other cancers
(including human tumors of the liver, lung, breast, and ovary) (62).
In vitro HSE-induced B16M-F10 cytotoxicity was very high (
90%)
when Bcl-2-AS-treated metastatic cells were treated with VRP
and ACV (Table VI). These results appear in agreement with
a recent report (63)
showing that GSH depletion enforces the mitochondrial permeability
transition and causes cell death in HL60 cells that overexpress
BCL-2. Furthermore, when BSO- and Bcl-2-AS-pretreated B16M-F10
cells were inoculated intravascularly into mice, the number of intact
arrested cells on the HSE decreased by
98%,
and the very small number of metastatic cell survivors (probably
bearing molecular damages) did not form detectable colonies (5).
Nevertheless, as indicated by the data displayed in Table
VII, after applying in vivo the
methodology proposed in this report, some metastatic cells may still
survive. Invasive cells may prolong survival under dormancy
conditions (64)
or may benefit from oxidative stress-promoting metastatic mechanisms,
e.g. increasing cell adhesion molecule expression (65),
activating early growth response-1 transcription factor gene (66),
activating metalloproteinases (67),
or increasing resistance to oxidative stress (68).
In fact, in mammalian cells at least 40 different gene products are
involved in adaptive responses to oxidative stress (68).
However, invasive B16M-F10 cells that survive after in vitro
interaction with the HSE show a transient impairment of the
mitochondrial system for GSH uptake (50).
The mitochondrial GSH is one of the endogenous effectors that
regulates the mitochondrial permeability transition pore complex (69),
and we observed that B16M-F10 cells with low mitochondrial GSH levels
were highly susceptible to TNF-
-induced
oxidative stress and death (50).
Most interestingly, this effect can be potentiated by Bcl-2-AS
therapy (5).
Moreover, therapy could be improved by combining nontoxic TNF-
doses with IFN-
(70),
or with a L-glutamine-enriched diet to
facilitate an L-glutamate-induced inhibition of GSH
transport into tumor mitochondria (71).
Furthermore, our methodology can be combined with cytotoxic drugs
and/or ionizing radiation. Thus the mechanisms described in this
report may have useful applications to improve therapy against
metastatic melanoma and, possibly, against other malignant tumor
types.
 |
FOOTNOTES |
* This work was supported by Grants
SAF2003-01886 and VIN01-013 from the Ministerio de Educación y
Ciencia and Grant GRUPOS03/185GVA from the Generalitat Valenciana
(Spain). The costs of publication of this article were defrayed in
part by the payment of page charges. This article must therefore
be hereby marked "advertisement" in accordance with 18 U.S.C.
Section 1734 solely to indicate this fact.
Recipient of a fellowship from the Ministerio de Educación
y Ciencia.
¶ Recipient of a fellowship from the
Generalitat Valenciana.
** To whom correspondence should be
addressed: Dept. of Physiology, Faculty of Medicine and Odontology, 17 Ave.
Blasco Ibańez, 46010 Valencia, Spain. Tel.: 34-963864646; Fax: 34-963864642;
E-mail: jose.m.estrela{at}uv.es
.
1 The abbreviations used are: B16M,
B16 melanoma; MRP, multidrug resistance protein; Bcl-2-AS,
Bcl-2 antisense oligodeoxynucleotide;
-GT,
-glutamyl transpeptidase; BSO, L-buthionine-(SR)-sulfoximine; DMEM, Dulbecco's
modified Eagle's medium; PMP, plasma membrane potential; CFTR, cystic
fibrosis transmembrane conductance regulator; G3PDH,
glyceraldehyde-3-phosphate dehydrogenase; VRP, verapamil; ACV,
acivicin;
-GCS,
-glutamylcysteine synthetase;
-GCS-HS,
-GCS heavy subunit;
-GCS-LS,
-GCS light subunit; HSE, hepatic sinusoidal endothelium;
LPS, lipopolysaccharide; PKA, protein kinase A; TUNEL, terminal dUTP
nick-end labeling; BCECF-AM,
2',7'-bis(2-carboxyethyl)-5,6-carboxyfluorescein acetoxymethyl
ester; PBS, phosphate-buffered saline; IFN, interferon; HSE,
hepatic sinusoidal endothelium; TNF, tumor necrosis factor;
ANOVA, analysis of variance.
 |
REFERENCES |
- Carretero, J., Obrador, E., Anasagasti, M. J., Martin, J. J.,
Vidal-Vanaclocha, F., and Estrela, J. M. (1999) Clin. Exp. Metastasis
17, 567-574[CrossRef][Medline]
[Order article via Infotrieve]
- Anasagasti, M. J., Martin, J. J., Mendoza, L., Obrador, E.,
Estrela, J. M., McCuskey, R. S., and Vidal-Vanaclocha, F. (1998)
Hepatology 27, 1249-1256[Medline]
[Order article via Infotrieve]
- Carretero, J., Obrador, E., Esteve, J. M., Ortega, A., Pellicer,
J. A., Sempere, F. V., and Estrela, J. M. (2001) J. Biol. Chem.
276, 25775-25782[Abstract/Free Full Text]
- Obrador, E., Carretero, J., Ortega, A., Medina, I., Rodilla, V.,
Pellicer, J. A., and Estrela, J. M. (2002) Hepatology 35, 74-81[CrossRef][Medline]
[Order article via Infotrieve]
- Ortega, A., Ferrer, P., Carretero, J., Obrador, E., Asensi, M.,
Pellicer, J. A., and Estrela, J. M. (2003) J. Biol. Chem. 278,
39591-39599[Abstract/Free Full Text]
- Estrela, J. M., Obrador, E., Navarro, J., Lasso De la Vega, M. C.,
and Pellicer, J. A. (1995) Nat. Med. 1, 84-88[Medline]
[Order article via Infotrieve]
- Zaman, G. J., Lankelma, J., van Tellingen, O., Beijnen, J.,
Dekker, H., Paulusma, C., Oude Elferink, R. P., Baas, F., and Borst, P. (1995)
Proc. Natl. Acad. Sci. U. S. A. 92, 7690-7694[Abstract/Free Full Text]
- Rappa, G., Lorico, A., Flavell, R. A., and Sartorelli, A. C.
(1997) Cancer Res. 57, 5232-5237[Abstract]
- Borst, P., Evers, R., Kool, M., and Wijnholds, J. (2000) J.
Natl. Cancer Inst. 92,
1295-1302[Abstract/Free Full Text]
- Gottesman, M. M., Fojo, T., and Bates, S. E. (2002) Nat. Rev.
Cancer 2, 48-58[CrossRef][Medline]
[Order article via Infotrieve]
- Zhou, D. C., Zittoun, R., and Marie, J. P. (1995) Leukemia
(Baltimore) 9, 1661-1666
- Meister, A. (1983) Science 220, 472-477[Medline]
[Order article via Infotrieve]
- Versantvoort, C. H., Broxterman, H. J., Bagrij, T., Scheper, R.
J., and Twentyman, P. R. (1995) Br. J. Cancer 72, 82-89[Medline]
[Order article via Infotrieve]
- Pommier, Y., Sordet, O., Antony, S., Hayward, R. L., and Kohn, K.
W. (2004) Oncogene 23, 2934-2949[CrossRef][Medline]
[Order article via Infotrieve]
- Ghibelli, L., Fanelli, C., Rotilio, G., Lafavia, E., Coppola, S.,
Colussi, C., Civitareale, P., and Ciriolo, M. R. (1998) FASEB J.
12, 479-486[Abstract/Free Full Text]
- Akerboom, T. P., and Sies, H. (1981) Methods Enzymol.
77, 373-382[Medline]
[Order article via Infotrieve]
- Martensson, J., and Meister, A. (1989) Proc. Natl. Acad. Sci.
U. S. A. 86, 471-475[Abstract]
- Eissa, S., and Seada, L. S. (1998) Clin. Chem. 44,
1423-1429[Abstract/Free Full Text]
- Lorico, A., Rappa, G., Flavell, R. A., and Sartorelli, A. C.
(1996) Cancer Res. 56, 5351-5355[Abstract]
- Miyake, H., Tolcher, A., and Gleave, M. E. (1999) Cancer
Res. 59, 4030-4034[Abstract/Free Full Text]
- Porteous, W. K., James, A. M., Sheard, P. W., Porteous, C. M.,
Packer, M. A., Hyslop, S. J., Melton, J. V., Pang, C. Y., Wei, Y. H., and
Murphy, M. P. (1998) Eur. J. Biochem. 257, 192-201[Abstract]
- Tata, F., Stanier, P., Wicking, C., Halford, S., Kruyer, H.,
Lench, N. J., Scambler, P. J., Hansen, C., Braman, J. C., Williamson, R., and
Wainwright, B. J. (1991) Genomics 10, 301-307[Medline]
[Order article via Infotrieve]
- Feinberg, A. P., and Vogelstein, B. (1983) Anal. Biochem.
132, 6-13[Medline]
[Order article via Infotrieve]
- Groen, A. K., Sips, H. J., Vervoorn, R. C., and Tager, J. M.
(1982) Eur. J. Biochem. 122, 87-93[Medline]
[Order article via Infotrieve]
- Estrela, J. M., Hernandez, R., Terradez, P., Asensi, M., Puertes,
I. R., and Vina, J. (1992) Biochem. J. 286, 257-262[Medline]
[Order article via Infotrieve]
- Cole, S. P., Sparks, K. E., Fraser, K., Loe, D. W., Grant, C. E.,
Wilson, G. M., and Deeley, R. G. (1994) Cancer Res. 54,
5902-5910[Abstract]
- Rosenfeld, H., and Roberts, J. (1981) Cancer Res.
41, 1324-1328[Abstract]
- Chomczynski, P., and Sacchi, N. (1987) Anal. Biochem.
162, 156-159[CrossRef][Medline]
[Order article via Infotrieve]
- Takada, A., and Bannai, S. (1984) J. Biol. Chem.
259, 2441-2445[Abstract/Free Full Text]
- Shaw, L. M., London, J. W., Fetterolf, D., and Garfinkel, D.
(1977) Clin. Chem. 23,
79-85[Abstract/Free Full Text]
- Aw, T. Y., Ookhtens, M., and Kaplowitz, N. (1984) J. Biol.
Chem. 259, 9355-9358[Abstract/Free Full Text]
- Aw, T. Y., Ookhtens, M., and Kaplowitz, N. (1986) Am. J.
Physiol. 251, G354-G361[Medline]
[Order article via Infotrieve]
- Meredith, M. J., Cusick, C. L., Soltaninassab, S., Sekhar, K. S.,
Lu, S., and Freeman, M. L. (1998) Biochem. Biophys. Res. Commun.
248, 458-463[CrossRef][Medline]
[Order article via Infotrieve]
- Gilbert, M. S., Saad, A. H., Rupnow, B. A., and Knox, S. J.
(1996) J. Cell. Physiol. 168, 114-122[CrossRef][Medline]
[Order article via Infotrieve]
- Wright, T. L., Fitz, J. G., and Boyer, T. D. (1988) Am. J.
Physiol. 255, 547-555
- Fernandez-Checa, J. C., Ren, C., Aw, T. Y., Ookhtens, M., and
Kaplowitz, N. (1988) Am. J. Physiol. 255, 403-408
- Liu, J. H., Miyakawa, H., Liu, J., Mori, K., Takano, T., Marumo,
F., and Sato, C. (1994) Res. Commun. Mol. Pathol. Pharmacol. 85,
261-270[Medline]
[Order article via Infotrieve]
- Linsdell, P., and Hanrahan, J. W. (1998) Am. J. Physiol.
275, 323-326
- Jungas, T., Motta, I., Duffieux, F., Fanen, P., Stoven, V., and
Ojcius, D. M. (2002) J. Biol. Chem. 277, 27912-27918[Abstract/Free Full Text]
- Gadsby, D. C., and Nairn, A. C. (1999) Physiol. Rev.
79, S77-S107[Medline]
[Order article via Infotrieve]
- McDonald, R. A, Matthews, R. P., Idzerda, R. L., and McKnight, G.
S. (1995) Proc. Natl. Acad. Sci. U. S. A. 92, 7560-7564[Abstract/Free Full Text]
- Cullen, K. V., Davey, R. A., and Davey, M. W. (2001) Biochem.
Pharmacol. 62, 417-424[CrossRef][Medline]
[Order article via Infotrieve]
- Loe, D. W., Deeley, R. G., and Cole, S. P. (2000) J.
Pharmacol. Exp. Ther. 293, 530-538[Abstract/Free Full Text]
- Tsuruo, T., Iida, H., Tsukagoshi, S., and Sakurai, Y. (1981)
Cancer Res. 41, 1967-1972[Abstract]
- Raderer, M., and Scheithauer, W. (1993) Cancer 72,
3553-3563[Medline]
[Order article via Infotrieve]
- Lu, S. C. (1999) FASEB J. 13, 1169-1183, and
references therein[Abstract/Free Full Text]
- Hanigan, M. H. (1995) Carcinogenesis 16, 181-185[Abstract]
- Moreadith, R. W., and Lehninger, A. L. (1984) J. Biol.
Chem. 259, 6222-6227[Abstract/Free Full Text]
- Stole, E., Smith, T. K., Manning, J. M., and Meister, A. (1994)
J. Biol. Chem. 269, 21435-21439[Abstract/Free Full Text]
- Ortega, A. L., Carretero, J., Obrador, E., Gambini, J., Asensi,
M., Rodilla, V., and Estrela, J. M. (2003) J. Biol. Chem. 278,
13888-13897[Abstract/Free Full Text]
- Dias, N., and Stein, C. A. (2002) Eur. J. Pharm. Biopharm.
54, 263-269[CrossRef][Medline]
[Order article via Infotrieve]
- Gleave, M. E., Miayake, H., Goldie, J., Nelson, C., and Tolcher,
A. (1999) Urology 54,
36-46[CrossRef][Medline]
[Order article via Infotrieve]
- Lopes de Menezes, D. E., Hudon, N., McIntosh, N., and Mayer, L.
D. (2000) Clin. Cancer Res. 6, 2891-2902[Abstract/Free Full Text]
- Klasa, R. J., Bally, M. B., Ng, R., Goldie, J. H., Gascoyne, R.
D., and Wong, F. M. (2000) Clin. Cancer Res. 6, 2492-2500[Abstract/Free Full Text]
- Zangemeister-Wittke, U., Schenker, T., Luedke, G. H., and Stahel,
R. A. (1998) Br. J. Cancer 78, 1035-1042[Medline]
[Order article via Infotrieve]
- Gross, A. (2001) IUBMB Life 52, 231-236[CrossRef][Medline]
[Order article via Infotrieve]
- Reed, J. C. (1997) Adv. Pharmacol. 41, 501-532[Medline]
[Order article via Infotrieve]
- Hickman, J. A. (2002) Curr. Opin. Genet. Dev. 12,
67-72[CrossRef][Medline]
[Order article via Infotrieve]
- Takaoka, A., Adachi, M., Okuda, H., Sato, S., Yawata, A., Hinoda,
Y., Takayama, S., Reed, J. C., and Imai, K. (1997) Oncogene 14,
2971-2977[CrossRef][Medline]
[Order article via Infotrieve]
- Dalton, W. S. (1993) in Cancer: Principles and Practice of
Oncology (De Vita, V. T., Jr., Hellman, S., and Rosenberg, S. A., eds) pp.
2655-2666, J. B. Lippincott, Philadelphia
- Hidalgo, M., Rodríguez, G., Kuhn, J. G., Brown, T., Weiss, G.,
MacGovren, J. P., Von Hoff, D. D., and Rowinsky, E. K. (1998) Clin. Cancer
Res. 4, 2763-2770, and references therein[Abstract]
- Hochwald, S. N., Harrison, L. E., Rose, D. M., Anderson, M., and
Burt, M. E. (1996) J. Natl. Cancer Inst. 88, 193-197[Abstract/Free Full Text]
- Armstrong, J. S., and Jones, D. P. (2002) FASEB J.
16, 1263-1265[Abstract/Free Full Text]
- Holmgren, L., O'Reilly, M. S., and Folkman, J. (1995) Nat.
Med. 1, 149-153[Medline]
[Order article via Infotrieve]
- Sellak, H., Franzini, E., Hakim, J., and Pasquier, C. (1994)
Blood 83, 2669-2677[Abstract/Free Full Text]
- Datta, R., Hallahan, D. E., Kharbanda, S. M., Rubin, E., Sherman,
M. L., Huberman, E., Weichselbaum, R. R., and Kufe, D. W. (1992)
Biochemistry 31, 8300-8306[Medline]
[Order article via Infotrieve]
- Lafrenie, R., Shaughnessy, S. G., and Orr, F. W. (1992) Cancer
Metastasis Rev. 11, 377-388[Medline]
[Order article via Infotrieve]
- Davies, K. J. (1999) IUBMB Life 48, 41-47[CrossRef][Medline]
[Order article via Infotrieve]
- Kroemer, G., and Reed, J. C. (2000) Nat. Med. 6,
513-519[CrossRef][Medline]
[Order article via Infotrieve]
- Sugarman, B. J., Aggarwal, B. B., Hass, P. E., Figari, I. S.,
Palladino, M. A., Jr., and Shepard, H. M. (1985) Science 230,
943-945[Medline]
[Order article via Infotrieve]
- Obrador, E., Carretero, J., Esteve, J. M., Pellicer, J. A.,
Pascual, A., Petschen, I., and Estrela, J. M. (2001) Free. Radic. Biol.
Med. 31, 642-650[CrossRef][Medline]
[Order article via Infotrieve]
- Yaziji, H., and Gown, A.M. (2003) Int. J. Surg. Pathol.
11, 11-15[Medline]
[Order article via Infotrieve]
Copyright © 2005 by the American Society for Biochemistry and
Molecular Biology.